Executive Summary
Vanadium storage and transport in amanita muscaria (fly agaric mushroom) and ascidians (sea squirts) dominate the discourse being had for the past 30 years on the bioinorganic chemistry of this very ubiquitos metal. Though the nuances and finer understandings of its mechanisms continue to evade scientists today, studies conducted within the past thirty years have shed light on what was a previously poorly understood metal. As such, elucidating vanadium’s importance in these two very unique organisms exemplifies the growing application of bioinorganic chemistry, small molecule models, and ligands to other realms of study such as drug delivery, insulin mimetics, and cancer studies.
Table of Contents
1. Vanadium: Characteristics & Versatility
2. Vanadium in Unique Organisms
3. Amavadin in Amanita Muscaria
4. Theorizing Transport & Storage in Ascidians (Sea Squirts)
5. Vanadium Transport
5.1. Vanabin2 as a transferase
1. Vanadium: Characteristics & Versatility
(Wikipedia source) Vanadium Metal Sphere
Vanadium is a soft, silver-colored metal with the electron configuration [Ar]4s23d3 and a molecular weight of 50.90 g/mol. It is a rather versatile metal, as demonstrated by its large range of oxidation states, V1- to V5, and ability to arrange itself in an abundant array of coordinate geometries, from one to eight. Although, V(V) to V(III) are the most biologically relevant oxidation states.
Vanadium plays a role in several contexts, below are just a few of many:
- Vanadium in Redox catalysis: Nitrogen fixation in the biosphere
The nitrogen cycle, the process by which atmospheric nitrogen (N2) exchanges with biologically active forms, such as those found in plants and many bacteria, is essential, and could not occur without vanadium. Exchange is crucial for all life, the buildup of excessive amounts of nitrogen in human beings can produce severe reactive nitrogen species (3).
The most important transfers and redistributions of reactive nitrogen among landscapes and waterscapes (see Glossary for abbreviations). Biological nitrogen fixation, denitrification, and a few minor transfers are omitted for visual simplicity (3).
- General Pathogenesis/Toxicity: Easy exchanges
Vanadium, as the vanadate species, is very similar to phosphate, which is essential to a host of metabolic enzymes to say the least (depicted top right). The physicochemical similarities between vanadate and phosphate make it possible for easy exchange to occur between the two and cause inhibition of a number of processes. Vanadium is ubiquitous in the environment, and about 10 μg to 2 mg are consumed per day between food and potable water. Saliva speciates vanadium into vanadyl hydroxide, VO(OH)2, and while most is excreted fecally, reabsorption of vanadium as vanadate and vanadyl does occur. The body reduces the presence of vanadium in the blood stream by about 30% every day. Though it can become trapped in bone to increase bodily half-life to about five days from uptake (2).
- Cancer Therapeutics: On the hunt for a cure
The field of cancer therapeutics is often searching for new compounds with the right chemical and physical properties to active cellular death pathways in cancerous cells, damage them, or inhibit key pathways they depend on to result in the same. Current research in this topic shows that vanadium complexes (ligands shown below) may have the right chemical and structural factors to induce apoptosis in cancer cells.
NN polypyridyl co-ligands and molecular structure of the oxidovanadium(IV) complexes with tridentate N-salicylidene-glycinato ligands. 1,10-phenanthroline (Phen, top right); 2,2’-bipyridine (bipy, top bottom); R- (Br or H) (6).
- Insulin Mimicry: Promising Diabetes Therapeutics
Vanadium has been found to imitate insulin function and thus play a role in treatment of diabetes. The square pyramidal compound, bis(maltolato)oxovanadium(IV) or BMOV (shown below) can increase glucose uptake. Interestingly enough, this ligand is a commonly a used food additive!
2. Vanadium in Unique Organisms
Some unique organisms, such as Amanita muscaria (red capped mushrooms) and Ascidians (sea squirts) have developed methods to uptake large quantities of vanadium and distribute it throughout their bodies. Researchers have hypothesized the uptake and storage of vanadium by these organisms to be defense mechanisms against predators. Though their specific biological role has yet to be determined.
Studies into both biological systems has allowed scientists to develop a working understanding of vanadium in living organisms. Current research explores coordination chemistry of protein carriers and complexes responsible for the uptake and storage of vanadium. The amavadin complex and vanabin family metallochaperones have been well studied and characterized in a mushroom (amanita muscaria) and sea squirts (ascidians), respectively.
3. Amavadin in Amanita Muscaria
The fly agaric mushroom (Amanita fungus), are red-capped mushrooms, and can be found in boreal forests, often living in colonies. These mushrooms are stationary, but they contain a special feature. On average, these fungi contain 400 times the normal amount of vanadium found in plants. Researchers have hypothesized the reason for such a high accumulation to be a defense mechanism against predators.
Amavadin is a dianionic complex with a molecular mass of 401.22 g/mole and can contain either of two different oxidization states of vanadium, Vv or VIV. A rare eight coordinate structure occurs with both cases. Complexes of both oxidation states contain few if any differences in their molecular structure, namely a few bond lengths. Amavadin has C2 symmetry, five chiral centers, and exhibits S stereochemistry.
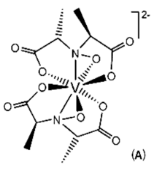
Image: Banks, C. H. [1]
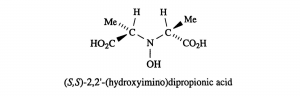
Image: Banks, C. H. [1]
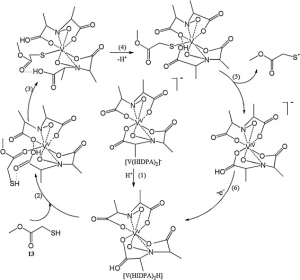
Image: Silva et al. [7]
4. Theorizing Transport & Storage in Ascidians (Sea Squirts)
Bioinorganic chemists understand vanadium to be essential or at least relevant still unsure as to the benefits and explicit mechanisms involving the metal. Various methodologies including but not limited to methodologies mentioned here have been implemented to further characterize this metal’s relevance to sea squirts.
K-edge X-ray absorption spectroscopy is an example of said methodologies. It is a technique that helps determine the local geometric and electronic structure of matter by shooting core electrons (not valence) with X-ray beams. “K” is a reference to the principal quantum n, which in this case represents the 1s shells and measures 1s to 3d excitation transitions. This is a perfect example of the utility of small ligand models. By using K-XAS, Frank et. al were able to intuit a proposed an binding/reductase enzyme, its reduction mechanism, active site structure, and make general statements about pH and specificity.
Vanadium K-edge XAS Absorption. Examining the various readings given off by exposing vanadium in sample vanadocytes to X-ray beams, scientists were able to match spectra similar to this sample to to the spectra of vanadium in a known complex and structure.
They did so by matching known models to experimental spectra. Fitting vanadium K-edge XAS spectra of whole vanadocytes with vanadium K-edge XAS spectra of appropriate models elucidated details of vanadium structure in blood of sea squirts under different conditions. They were able to make the inference that different incubation conditions induced production different bound form of vanadium, thereby drawing conclusions about relevant vanadium coordinations numbers, pH dependency, and general specificity of this enzyme [7].
Here is resultant complexation and reduction of V(III) within the vacuole of vanadocytes of ascidians proposed by Frank et. al. K-edge XAS data suggests that the geometric and electronic arrangement of ligands around vanadium resemble that of vanadium complexed in an EDTA-like environment. (EDTA coordination number, 7).
Immunological Techniques such as Western blotting actually elucidated the existence of possible transporters in membranes of these organisms which ferry vanadium in and out of blood cells. The image to the right is of a gel run of this nRamp protein (discovered 2010 by Ueki et. al) by bound to an antiRamp antibody and the gel separation and fractionation that followed.9 This nRamp protein was fractionated in the same fraction as another large, V2C12 protein. Similar to V2C12, its apparent MW was higher than the expected value which is presumed to be due to glycosylation, a characteristic of membrane proteins. These results suggested that AsNramp was localized on the vacuolar membrane of the blood cells of the ascidians. Since the pH of the vacuole is extremely low in vanadium-rich ascidians, we expected that AsNramp could act as proton-dependent vanadium transporter. AsNramp mediates vanadium accumulation coupled with the electrochemical gradient generated by vacuolar H+-ATPase in vanadocytes.
What we know from these past methodologies and experiments: Vanadium is being ferried by some unknown binding proteins, traveling through certain membrane-bound transporters, and being reduced from V to III in the process until they reach the vacuole, where they are ultimately stored in a low pH environment. Let’s dive deeper!
5. Vanadium Transport
In Ascidians, of which there are from 900 to 1000 species, vanadium can accumulate to a concentration that is 107 greater than the environment, and is doubly oxidized from VV to VIII, from uptake to final storage, respectively. A chematic of vanadium uptake and storage in ascidians is depicted to the left (9).
Sea water containing vanadyl hydroxide species makes its way from the mouth to brachial sac or coelum (only present in some species). From this point, vanadium species can move from the digestive tract into the bloodstream. It is currently not know whether or not there is a protein mediating diffusion across these tissues.
Once in the bloodstream, vanadyl species are uptaken by vanadocytes, cells uniquely in charge of the uptake and storage of the vanadium in ascidians. Some species of sea squirts, such as ascidia sydneiensis samea, contain proteins such as vanabinP and VBP-129, responsible for the transport of vanadate species in the bloodstream to the vanadocytes. Yet to be understood is how the actual uptake of vanadium species into vanadocytes occurs. Given the similar nature of vanadate to phosphate, one hypothesis presents a phosphate transmembrane protein as the mediator of uptake. Another idea presents the possibility that a protein similar to transferrin (responsible for iron uptake) is responsible.
It is clear that once in the the cytoplasm of a vanadocyte, vanadate species are sequestered by the vanabin 2. The species are bound, reduced from the VV to VIV oxidation state, and taken to a vacuole (a membrane enclosed space) for storage. The NRAMP antiporter is responsible for uptake of the vanadium species while an ATPase responsible for uptake of protons, thereby lowering the pH of the vacuole. Under extremely acidic conditions, pH 2, and in the presence of sulfate species, VIV reduced to the VIII oxidation state (2,5,6,8-10).
5.1 Vanabin2 as transferase
Vanabin 2, functional in the vanabin family of metallochaperones, deals with V uptake; transporting cytosolic vanadium species to the vacuole. It is a small molecular weight protein, 15 kD, with 4 four alpha helices containing 18 cysteine residues (ribbon structure shown left, 5). These residues are able to form nine disulfide bonds that are essential to protein structural stability and vanadium binding ability.
Binding of vanadium species has been shown to be localized to the same face of the protein, the inwardly curved, central region. Coordination occurs via amine-N donors such lysine, arginine, and histidines. Around ten to twenty ions (VIV) are bound at a time, with a Kd of about 2×10-5 M. Under acidic conditions, vanabin 2 is also able to bind iron(III) and copper(II).
5.2 Vanabin2 as reductase
Vanabin2 ferries vanadium in the signet blood cells (vanadocytes) of sea squirts. It is also a major reducing agent, catalyzing the reduction of VV to VIV in the cytoplasm of the vanadocyte. It does so by coupling metal reduction to the oxidation of NADPH, a ubiquitous electron carrier across many species of organisms. The image below shows the hypothesized mechanism around the vanabin2 active site. H2VO4 (natural form of vanadium at a neutral pH) complexes with the enzyme (accompanied by a loss of H2O) wherein carboxylates and nitrogenous side chains become ligands.
The outward curvature of the protein indicates that the majority of the structure of the enzyme is unknown save for the active site. The bracketed N’s indicate nitrogens from amino acids residues such as lysine and arginine with nitrogenous side chains.
The literature suggests that electrons may be transferred from the NADPH to acceptor VV ions via thiol-disulfide exchange rxns (depicted below). Specifically, vanabin 2 catalyzes the reduction oxidation of NADH to NADP+ via reduction of glutathione reductase (GR). Afterwards, the oxidized form of glutathione (GSSG) can be reduced by GR, to then transfer those electrons to cysteinyl residues of vanabin 2. These electrons can be transferred to the active site and reduce VV to VIV. This is is perfect example of a double ping-pong reaction.
6. Online Resource
Rundown of Vanadium Chemistry in Amanita Muscaria and Ascidians!
This video, created by the Professor D. Ray at the Indian Institute of Technology (in association with the National Program on Technology Enhanced Learning) provides a detailed visual explanation of vanadium chemistry as it pertains to the fly agaric mushroom and sea squirts. It provides a detailed picture of vanadium storage and transport of what is known now. He seems to be referencing some papers and visuals that you will also find referenced below (Turn down for what?).
Congrats! You Know Vanadium Chemistry!
7. References
- Banks, C. H., Vanadium. In Vanadium-Chemistry Encyclopedia, 2017.
- Costa Pessoa, J.; Garribba, E.; Santos, M. F. A.; Santos-Silva, T., Vanadium and proteins: Uptake, transport, structure, activity and function. Coordination Chemistry Reviews 2015, 301–302, 49-86.
- Davidson, E. A. D., Mark B.; Galloway, James N.; et al., Issues in Ecology Ecological Society of America 2012.
- Frank, Patrick, Elaine J. Carlson, Robert M. K. Carlson, Britt Hedman, and Keith O. Hodgson. “The Uptake and Fate of Vanadyl Ion in Ascidian Blood Cells and a Detailed Hypothesis for the Mechanism and Location of Biological Vanadium Reduction. A Visible and X-Ray Absorption Spectroscopic Study.” Journal of Inorganic Biochemistry 102, no. 4 (2008): 809–23. Accessed February 27, 2017. doi:10.1016/j.jinorgbio.2007.12.001.
- Redher, D., The role of vanadium in biology. Metallomics 2015, 7, 730-742.
- Scalese, G.; Correia, I.; Benítez, J.; Rostán, S.; Marques, F.; Mendes, F.; Matos, A. P.; Costa Pessoa, J.; Gambino, D., Evaluation of cellular uptake, cytotoxicity and cellular ultrastructural effects of heteroleptic oxidovanadium(IV) complexes of salicylaldimines and polypyridyl ligands. Journal of Inorganic Biochemistry 2017, 166, 162-172.
- Silva, J.A.L; Frausto, J.J.R. “Amavadin: A Vanadium Natural Complex: ITs role and Applications.” Coordination Chemistry Reviews. Vol. 257, 15-16 (2013), 2388-2400. Accessed FFebruary 27, 2017. doi.org/10.1016/j.ccr.2013.03.010
- Ueki, T.; Michibata, H., Molecular mechanism of the transport and reduction pathway of vanadium in ascidians. Coordination Chemistry Reviews 2011, 255 (19–20), 2249-2257.
- Ueki, Tatsuya, Nobuaki Furuno, and Hitoshi Michibata. “A Novel Vanadium Transporter of the Nramp Family Expressed at the Vacuole of Vanadium-Accumulating Cells of the Ascidian Ascidia Sydneiensis Samea.” Biochimica et Biophysica Acta (BBA) – General Subjects 1810, no. 4 (2011): 457–64. Accessed February 27, 2017. doi:10.1016/j.bbagen.2010.12.006.
- Ueki, Tatsuya, Nobuo Yamaguchi, Romaidi, Yoshiaki Isago, and Hisashi Tanahashi. “Vanadium Accumulation in Ascidians: A System Overview.” Coordination Chemistry Reviews 301-302 (2015): 300–308. Accessed February 27, 2017. doi:10.1016/j.ccr.2014.09.007.