Quantum mechanical phenomena are in general impossibly difficult to simulate on an ordinary computer. To see why, consider the simplest quantum system imaginable: a single particle with two states which we might label |0> and |1>. For an arbitrary state
|Ψ> of this system we might write
|ψ> = α|0> + β|1>,
i.e. there are two `weights’ α and β which describe the probability that we might ultimately find our particle in |0> or |1>, respectively, if we were to measure it. So, we need two weights to write down our general state |ψ>. Simple enough, but it turns out that things get more complicated if we add particles to our system. If we had three of these particles and measured their state, we might find all three to be in the state |0>, which we might write as |000>. But, we might also measure |001>, or |010>, or any of the other possible combinations – there are eight possibilities in total. So, to write down the general state of the system we would now have to write
|ψ> = α|000> + β|001> + γ|010> + . . . + θ|111>;
|ψ> now spans a space with eight `basis states,’ and eight weights are needed to write it down. More generally, for N particles there are 2N weights. As a result, calculations involving quantum systems slow down exponentially with system size.
Fortunately, quantum mechanics offers some alternative ways to circumvent the problem. In the Doret lab we are interested in analog quantum simulation, wherein one quantum system is used to emulate the interactions and behavior of a second system which is challenging or impractical to study in its native form.
Trapped Ion Quantum Simulation
Atomic ions are a good choice to use as the `engine’ for such a quantum simulator. The ions’ charge gives us a handle with which to hang on to them in electromagnetic traps, and laser or microwave fields can be used to manipulate and control the ions’ states. Our goal is to perform simulations based around the vibrational degrees of freedom of the transverse motion of a chain of trapped ions.
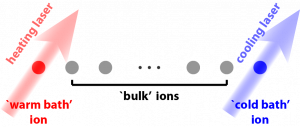
Ion configuration for studying heat flow.
As one example, we are working towards an investigation of thermal conductivity at mesoscopic scales such as those found in modern digital electronics. By using laser-cooled ions to implement thermal reservoirs at each end of a chain of ions we plan to measure the flow of energy along the chain. We ultimately plan to tailor the interaction between these ions’ vibrations and their internal states to simulate disordered materials and study the onset of Fourier’s Law thermal gradients at the nanoscale. Along the way we plan to study methods for improving the cooling rates of slowly cooled modes in sympathetically cooled ion chains, a problem likely to be critical developing large-scale trapped ion quantum information processors in the years to come.
Precision Measurement
Trapped ions are also a wonderful system for pursuing precision measurements; few systems do a better job of approximating the ideal of a single, isolated atoms free of external perturbations. Measurements in systems of trapped ions are thus central to improvements in precise metrology, searches for New Physics, and probing atomic and nuclear structure.
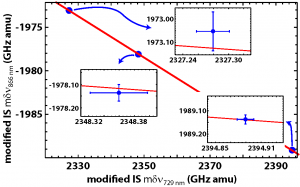
2D King Plot of the Ca+ 866 nm transition isotope shifts (from PRL 115, 053003 (2015)) against our recent 729 nm E2 transition isotope shift measurement
Motivated in part by our plans to use electric quadrupole transitions for `thermometry’ in our planned study of the onset of Fourier’s Law, our lab has been pursuing precise measurements of isotope shifts in forbidden transitions in Ca+. These shifts are central to determinations of nuclear charge radii. Additionally, isotope shifts for different transitions in the same set of nuclei are linearly related to first-order. Searches for non-linearities in this relationship are sensitive to both to Standard Model physics such as nuclear polarizabilities and possible new, Beyond Standard Model interactions.
Laboratory Setup
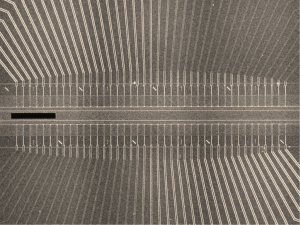
`Gen V’ ion trap from GTRI
Our experiments take place within a surface electrode ion trap designed and fabricated by the Georgia Tech Research Institute. Surface electrode traps typically feature many control electrodes located close to the trapped ions, enabling precise tailoring of confining potentials and trapping of ions in multiple zones of the trap. Our trap has approximately ninety electrodes, each individually controlled.
To load and trap ions we resistively heat an oven loaded behind the traps `loading slot’ to produce a beam of neutral calcium ions. These ions stream through the slot and can then be photoionized and trapped by a combination of static and oscillating electric fields. The trap is maintained under ultra-high vacuum in a stainless steel chamber with viewports that permit laser access over the trap surface. A large, home-built lens collects approximately 5% of the light emitted by the ions and images it onto a CCD camera and a PMT.

Schematic view and photograph of our trap setup.
Trapping calcium ions requires a number of lasers for photoionization, Doppler cooling, state preparation, and coherent manipulation. One of the nice features of calcium is that all essential transitions are at wavelengths for which optical fibers and laser diodes are readily available. We thus use home-built lasers and control electronics throughout the lab, including a simple, very low noise piezo driver of our own design. To ensure our lasers remain locked to the correct wavelengths we use a scanning Fabry-Perot cavity to transfer the stability of a commercial stabilized HeNe onto our diode lasers. Acousto-optic modulators can then be used to control the frequency, intensity, and duration of laser pulses used to cool, manipulate, and interrogate the trapped ions.
Student Research Participation
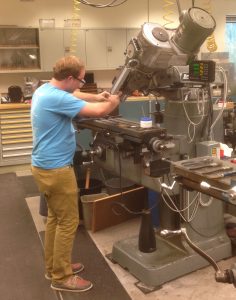
Cole Meisenhelder ’15 in the shop
At Williams undergraduate researchers play a central role in moving research projects forward. This is especially so in the Doret lab; with so much of our equipment has been home-built, students are involved with every aspect of the work ranging from designing and assembling the tools we use to taking data in the laboratory. To offer a few examples from recent thesis projects:
- Felix Knollman ’19 has been hard at work collecting and analyzing data associated with our 729 nm E2 transition isotope shift measurement.
- Ashay Patel ’18 characterized and compensated for the stray field environment in our trap using a four-channel frequency source he built to control our AOMs. He also developed new trap voltage waveforms for merging & splitting chains of ions.
- Sierra Jubin ’17 assembled our vacuum chamber and trap control hardware, supervised the bakeout of the chamber, and trapped our first ions.
- Sarah Stevenson ’17 developed an optical setup for frequency stabilizing and linewidth narrowing a 729 nm laser using a ULE reference cavity.
- Ariel Silbert ’16 designed and assembled the helical resonator we use to drive the oscillating potentials on our ion trap and also developed software we use to control our trap electrodes.
- Cole Meisenhelder ’15 did extensive work developing our diode laser design, including fabricating components in the college machine shop for mounting the optics and building two complete sets of control electronics.
Quite a few additional students have played crucial roles in supporting these thesis projects, including work designing printed circuit boards, building radio-frequency amplifiers, aligning optics, and writing software. Opportunities abound for students at any point in their education ranging from first-year students to seniors completing honors research theses. To learn more, take a look at the People page to check out student theses, stop by the lab in SSB 003, or visit with Professor Doret.